19 minute read
Rujuta Purohit '24
Supermassive Pockets in the Cosmic Neighborhood: Searching for Active Galactic Nuclei
BY RUJUTA PUROHIT '24
Cover Image: The spiral galaxy NGC 3393 hosts an obscured active galactic nucleus at its center. In the sky, NGC 3393 is located in the constellation of Hydra and is about 56 mega parsecs away from us. The galaxy is characterized as a Seyfert galaxy and actually has two supermassive black holes at its core.
Image Source: Wikimedia Commons
Introduction
An active galactic nucleus (AGN) is the physical manifestation of gas accreting onto a supermassive black hole (SMBH). SMBHs lie at the centers of most, if not all, large spiral and elliptical galaxies and are believed to be responsible for powering the galaxy. Sagittarius A* (Sgr A*) is the SMBH at the center of the Milky Way Galaxy. Although not producing large amounts of radiation (thus, often called dormant) today, the SMBH is truly giant, with a mass of 4.3 million times that of the Sun (Abuter, 2019). In 2020, Andrea Ghez of the University of California, Los Angeles, and Reinhard Genzel of the Max Planck Institute received the Nobel Prize in Physics for proving that Sgr A* indeed hosts a supermassive black hole at its center.
SMBHs have masses on the order of millions of times that of the Sun and are very strong sources of radiation. Their strong gravitational force means that no physical matter can escape their event horizons, including light. Event horizons are the last optically visible regions of the black holes themselves; they are large, compact discs of gas and dust surrounding the center of a black hole. The event horizon is what could theoretically be “seen” when looking at a black hole.
At any given time, the majority of SMBHs are mostly dormant at the centers of their host galaxies. However, a small fraction is known to emit significant amounts of light and energy. This is due to an interesting combination of many factors, including the masses of the black holes and their host galaxy, the rate of star formation in the galaxy, and the amount of dust and gas present in the vicinity of the SMBH. These black holes then outshine the entire host galaxy across the electromagnetic spectrum, resulting in an active galactic nucleus (AGN). AGN are powered by the release of gravitational energy from all of the matter that is accreted onto the SMBHs. The large energy released by an AGN can effectively influence its surrounding environment, eventually impacting the whole nuclear region, as well as the star formation and the evolution of the galaxy as a whole (Masini et al., 2020).
Physical Characteristics of AGN
The Eddington luminosity (also called the Eddington limit) is the maximum luminosity an object can ever have such that the inward gravitational force is balanced by the outward radiation force. If the luminosity exceeds the Eddington limit, the radiation pressure drives jets, which are high-energy beams of matter travelling out of the center of the black hole at relativistic speeds (speeds very close to that of light) (Bassani & Sembay, 1983). They are extremely energetic and thus very bright. The jets
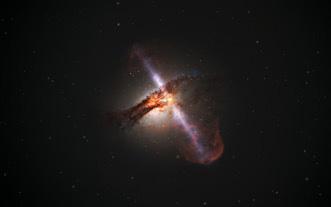
Image 1: An artist’s illustration of relativistic jets being ejected from the core of a supermassive black hole of an active galaxy. The jets are often thousands of parsecs long and are very energetic. Image Source: Wikimedia Commons
have lengths that range from anywhere between a few thousand parsecs to millions of parsecs (Kundt, 2015). It is unknown what exactly causes the jets, but it is believed that they result from electron-positron interactions at high velocities (Georganopoulos et al., 2005). Figure 1 shows an artist’s illustration of a spiral galaxy with jets from a supermassive black hole.
The accretion of gas around the SMBH produces an optically thick disk of material, called the accretion disk. The accretion disk emits thermally because of the viscosity within the disk. The gas within the accretion disk has a wide range of temperatures, and the emission is consequently produced over a broad wavelength range (Gurzadyan, 1979).
AGN are interesting astronomical phenomena since they are regions and not objects themselves. The physical model of an AGN thus hopes to describe the many features of the black hole and the galaxy. There are a number of theories that seek to model the physical structure of AGN. The currently accepted model is called the Unified Model of the AGN and explains the structure by dividing the AGN region into 6 main regions: the black hole, the accretion disk, the torus, the narrow-line region, the broad-line region, and the outflow (jets) (Hickox & Alexander, 2018). Jets and accretion disks are described above but will be discussed again in context of the model.
The accretion disk is surrounded by a geometrically and optically thick dusty and molecular torus, often referred to as the dusty torus. It is thought to be within the gravitational influence of the SMBH. The torus is composed of the cooler, outer regions of the accretion disk where molecules and dust grains can form (Hickox & Alexander, 2018). The torus is broadly symmetric around the accretion flow axis.
The narrow-line region (NLR) and the broadline region (BLR) are defined on the basis of the velocity width of the emission lines obtained for the radiation of the AGN. The BLR has gases with a broader distribution of emission line velocities than the NLR. The typical range of velocity widths for the gas in the BLR is about 1,000 – 10,000 km/s while that for the NLR it is about 100 – 500 km/s (Padovani et al., 2017). The different velocity widths of the BLR and NLR result from the relative location of the gas with respect to the SMBH.
The gas in the BLR lies within the gravitational influence of the SMBH and thus resides close to the accretion disk. By comparison, the gas in the NLR lies under the gravitational influence of the host galaxy and is produced on larger scales. The bulk of NLR emission generally originates within the central kiloparsecs, but for some systems the emission from the gas ionized by the AGN is observed on the scale of the entire galaxy, out to ≈ 10 kilo parsecs (Hainline et al., 2014).
The radiation from the AGN impinges on the interstellar medium in space, exciting the gas by ionization and transferring mechanical energy to the gas through radiation pressure and disk winds.
Image 2: A sketch of the main AGN structures under the Unified Model of the AGN. The sketch shows the SMBH, the accretion disk, the torus, the BLR, the NLR, and the outflow.
Image Source: (Hickox & Alexander, 2018)
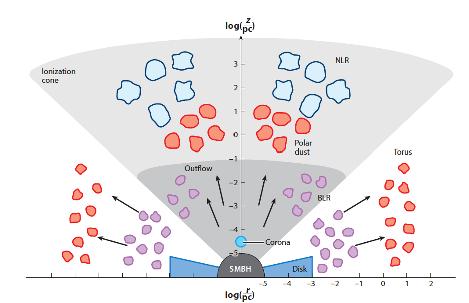
This appears in the form of cones extending from the nucleus (Durre & Mould, 2018). Figure 2 shows a sketch of the main AGN structures seen along the equatorial and polar directions. The regions are colored according to their contributions to the SED in Figure 3 that follows.
Searching for AGN
A term useful when searching for AGN is the “bolometric luminosity.” The bolometric luminosity of an AGN corresponds to the total luminosity produced by the accretion disk. It is a measure of the luminosity produced across the entire electromagnetic spectrum.
Temperatures are also useful in determining what physical properties are needed to search for AGN and what regions of the EM spectrum they can be assigned to. For the accretion disk of a typical AGN, the range of gas temperatures is likely to be T about 104 K, and therefore the majority of the emission from the accretion disk will be at detected between 30 and 300 nm (i.e., at UV–optical wavelengths) (Shields, 1999). A plot of the emission fluxes against the different wavelengths is called the spectral energy distribution (SED). SEDs are relatively unique for different astronomical objects as fluxes are representative of an object’s mass and size. The SED of an AGN accretion disk is distinct from the SEDs of other astrophysical sources and is comparatively easy to identify. A SED is thus one of the first ways to identify an AGN. Figure 3 is the SED of an AGN with different wavelength ranges indicated for different wavelengths.
Different methods are employed in different bands to identify the AGN sources. The various wavelength regimes provide different windows on AGN physics. Namely, the infrared (IR) band is mostly sensitive to dust, the optical/ ultraviolet (UV) band is related to emission from the accretion disk, and the X-ray band traces the emission of a corona ��-ray. High flux density radio samples, on the other hand, preferentially select AGN emitting strong non-thermal jetrelated radiation (Padovani, 2017).
Image 3: : The spectral energy distribution of an AGN. The horizontal axes show the wavelength in two different scales and the vertical axis shows the luminosity of the AGN over those wavelengths. Image Source: (Hickox & Alexander, 2018)
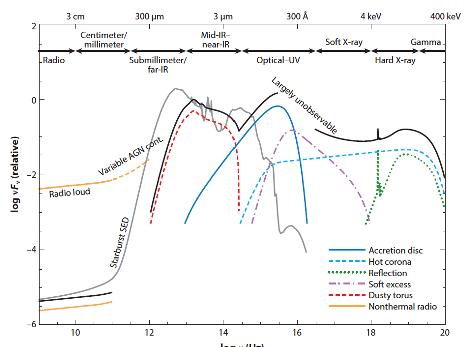
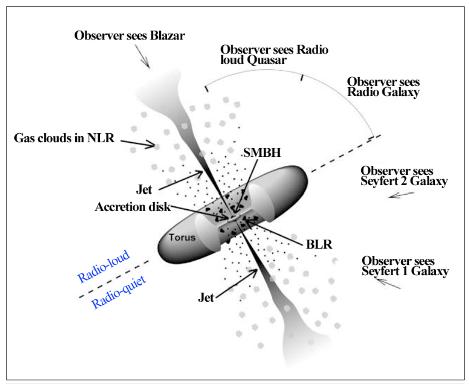
There are two broad categories into which AGN are classified: radio-quiet AGN and radio-loud AGN. Like the name suggests, radio loud AGN have emissions from jets and the jets’ lobes. The emissions of radio quiet AGN can be neglected since they are not strong enough to always be detected. Within these two categories, there are lots of types of AGN (Komberg, 1992).
Radio-quiet AGN include low-ionization nuclear emission-line regions (LINERs), Seyfert galaxies, and radio-quiet quasars. LINERs have weak emissions and are thought of as “maybe AGN”. They are hard to detect and classify with certainty, since the weak nuclear emission isn’t supported by other emission lines (Belfiore, 2016).
Radio galaxies are AGN that are very luminous at radio wavelengths. The observed structure in radio emission is determined by the interaction between the jets from the galaxies and the gas/ dust. Most radio loud AGN are ellipticals (Kraft, 2003).
Seyfert galaxies are detected using their optical range nuclear continuum emission. These AGN have very high surface brightness and reveal high-ionization emission lines. As a result, their ionization cones are very large and distinct. The host galaxies are easily detectable using the emission lines. These galaxies have bolometric luminosities on the order of 1042 – 1045 ergs/s (Hickox & Alexander, 2018). Seyfert galaxies show an excess of radiation in the far infrared region of the EM spectrum. At some wavelengths, this excess radiation is variable, and it is thus theorized that the excess variable radiation actually comes from the compact AGN inside the galaxy. Quasars— “quasi-stellar radio-source objects”— are more luminous Seyfert galaxies. Quasars produce large amounts of radiation and are thought to be one of the most luminous objects in the entire Universe; a quasar alone could potentially emit energy that is a thousand times more than that of the entire Milky Way Galaxy (Wu, 2015). Quasars have bolometric luminosities greater than 1045 ergs/s (Hickox & Alexander, 2018). Since they are extremely luminous and powerful, it is believed that they were more common in the early Universe. Energy production would slow down and come to a halt later in the lifetime of an active galaxy. This is used to support the thesis that most large galaxies go through an active phase (Tiziana et al., 2005). For example, the Milky Way is no longer an active galaxy, but the black hole in its center might have once been an AGN.
Understanding the nature of AGN better is intricately linked to our understanding of the conditions of the early universe, black-hole accretion models, and the theories surrounding the Big Bang. Furthermore, since these were more common in the early Universe, measuring quasar redshift could help create better models for the expansion of the Universe. A large redshift would mean that the quasar is far away from the Earth – if a measured redshift is due to the expansion, then such objects’ high luminosity could only be explained by high initial masses. Such conditions would only be possible if they were formed in the early Universe. Following this cyclic reasoning, quasars can only be as luminous and powerful as they are now if there were formed at the center of and right at the beginning of the Universe where they could accrete more mass and then move father away due to the expansion (Grupen, 2005). In contrast, Seyfert galaxies are closer to the Earth as compared to quasars and are believed to have formed later in the evolution of the Universe.
Image Source: NASA Open Source
"Understanding the nature of AGN better is intricately linked to our understanding of the conditions of the early universe..."
Image 5: NGC 1448, a galaxy with an obscured AGN, is seen in this image combining data from the Carnegie-Irvine Galaxy Survey in the optical range and NuSTAR in the X-ray range. Image Source: NASA Open Source
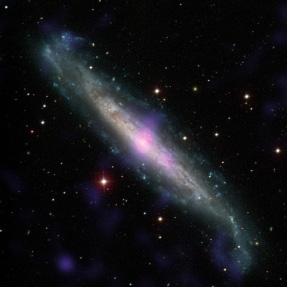
sources of high-energy gamma ray photons. The jets extend up to 100 kiloparsecs from the central black hole (Kellermann, 1992). The observed emission from a blazar is greatly enhanced by relativistic effects in the jet, a process called relativistic beaming. The relativistic jets characteristic of blazars are such that they are oriented close to the line of sight of the observer from the Earth.
The accretion of gas onto a SMBH is an exceptionally efficient process. About 5% to 42% of the mass is ultimately converted into emission, depending on the spin of the SMBH (Shapiro & Teukolsky 1983), and therefore large luminosities can be produced for a modest amount of accretion, allowing for luminous AGN to be detected out to high redshifts. Most AGN are detected by searching for such redshifts over different luminosity values.
Figure 4 shows a schematic of the physical regions of an AGN and how they get classified between the different types depending on the physical characteristics that are detected.
Obscured Active Galactic Nuclei
Although they are enormous and have great gravitational influences, active galactic nuclei cannot always be easily detected. The huge radiative power of most AGN cannot be seen directly, since the accretion is hidden behind gas and dust that absorb many of the characteristic observational signatures (Hickox & Alexander, 2018).
Obscuration is defined as anything that absorbs emission and/or scatters a large fraction of it away from the line of sight of the observer. In astrophysical sources, the obscuring medium is typically composed of dust and/or gas. Broadly, dust particles are solid state structures which are typically carbonaceous grains and amorphous silicate grains, in UV-IR wavelengths. Gas molecules are just gaseous states particles, ranging from fully ionized gas including electrons and protons, to neutral gas and molecular compounds. For many of, and probably the majority of, obscured AGN, the obscuration occurs in the close vicinity of the accretion disk and lies within the gravitational influence of the SMBH (Hickox & Alexander, 2018).
The impact of the obscuring material on the detection of the accretion disk emission is directly dependent on the wavelength used for observation. This is called the optical depth. It is a measure of absorptivity up to a specific depth (or length of distance) across space. A small optical depth means that a small fraction of the emission will be absorbed, whereas a large optical depth means that a large fraction of the emission will be absorbed (Hickox & Alexander, 2018). The optical depth is one of the most important factors in determining whether an AGN is obscured or not.
In an AGN, the observed X-ray emission is absorbed by material in the surrounding torus. Any obscuration also always occurs in close vicinity of the e accretion disk and lies within the gravitational influence of the SMBH (Hickox & Alexander, 2018). The radiation is then re-emitted at mid-infrared wavelengths by the torus. Thus, the X-ray and mid-infrared luminosities of AGN are related through a defined and predictable relationship. If an AGN has a mid-IR luminosity that is greater than the X-ray luminosity, then the AGN is said to be obscured (Lambrides et al., 2020). These AGN are often found in the faintest group of observations which makes them even harder to detect. Galaxy NGC 1448, shown in Figure 5, hosts an obscured AGN at its center.
The classical definition of an obscured AGN is the absence of emission from the BLR in the optical waveband (Antonucci, 1993). This corresponds to a typical obscuring screen or extinction from dust. For typical dust-to-gas ratios, this corresponds to an equivalent absorbing column density from gas measured in the X-ray band of NH > 1022 cm−2. The NLR can be detected in both obscured and unobscured AGN, whereas the BLR is expected to be detected only in unobscured AGN. Because of its extent and its positioning, the emission from the NLR is generally not obscured (Hickox & Alexander, 2018).
Certain obscured AGN are called “Comptonthick” AGN. Compton-thick active galactic nuclei (CTAGN) are defined as AGN that are obscured along the line-of-sight by absorbing gas with a column density of NH 1024. The study of Compton-thick AGN is crucial and will enlarge our current database for obscured AGN. Observational evidence suggests that a large fraction of AGN in the local universe are obscured by Compton thick gas (Matt et al., 2000). The absorbed luminosity eventually will be reemitted in the far-infrared (far-IR) by the accretion disk makes. This makes Comptonthick sources potential contributors to the long wavelength radiation emitted by some AGN. Finally, accretion in the Compton-thick AGN may contribute to the local black hole mass density and help create better models for the formation of black holes.
The search for Compton-thick AGN has been limited to the relatively bright fluxes accessible to the high energy detectors. Careful analysis of X-ray observations can help find such sources, with Mrk 462 being one of the very few Comptonthick obscured AGN to be detected in a dwarf galaxy. Recent research has focused on identifying and studying obscured AGN, especially those in low mass galaxies since their numbers are poorly defined. It is believed that this will place time and mass constraints on the evolution of galaxies and the formation of black holes in these galaxies (Parker & Hickox, 2021).
Conclusion
Supermassive black holes are found at the centers of most massive galaxies and are thought to be their sources of power. The accretion of gas onto such a black hole is called an active galactic nucleus (AGN) that emits radiation over many different wavelengths. The Unified Model of the AGN describes various regions of the AGN like the accretion disk, the torus, the outflow (jets), the narrow-line region, and the broad-line region. These physical regions and how they are detected are used to classify AGN. There are two main types of AGN: radio-quiet and radio-loud. These have further subtypes with unique characteristics and offer information about the formation and evolution of the Universe.
The majority of the AGN population is obscured by the dust and gas surrounding it. Therefore, the construction of a complete census of AGN activity requires the identification of both obscured and unobscured sources. A complete census of AGN activity is required to reliably measure the cosmological buildup of SMBHs and to place fundamental constraints on the efficiency of SMBH growth (Hickox & Alexander, 2018). Such a census would also prove helpful in analyzing the influence SMBHs have on powering the host galaxy.
The occupational fraction of lower mass black holes in dwarf galaxies is poorly constrained; searching for such black holes requires greater spatial resolution than is afforded by most intermediate mass black holes. It is believed that detecting more obscured AGN in general and more obscured dwarf AGN will greatly add to current theories on galaxy evolution and black hole formation.
References
Abuter, R., Amorim, A., Bauböck, M., Berger, J. P., Bonnet, H., Brandner, W., ... & Yazici, S. (2019). A geometric distance measurement to the Galactic center black hole with 0.3% uncertainty. Astronomy & Astrophysics, 625, L10.
Antonucci, R., Kinney, A. L., & Hurt, T. (1993). “Hubble Space Telescope ultraviolet spectroscopy of the highly polarized but quiescent quasar OI 287”. The Astrophysical Journal, 414, 506-509.
Bassani, L., Dean, A. J., & Sembay, S. (1983). “Super-Eddington luminosity characteristics of active galactic nuclei”. Astronomy and Astrophysics, 125, 52-58.
Belfiore, Francesco (2016). "SDSS IV MaNGA - spatially resolved diagnostic diagrams: a proof that many galaxies are LIERs". Monthly Notices of the Royal Astronomical Society. 461 (3): 3111. arXiv:1605.07189. Bibcode:2016MNRAS.461.3111B. doi:10.1093/ mnras/stw1234 "...studying obscured AGN...will place time and mass constraints on the evolution of galaxies and the formation of black holes in these galaxies..."
Durré, M., & Mould, J. (2018). “The AGN Ionization Cones of NGC 5728. I. Excitation and Nuclear Structure”. The Astrophysical Journal, 867(2), 149.
Georganopoulos, M.; Kazanas, D.; Perlman, E.; Stecker, F. W. (2005). "Bulk Comptonization of the Cosmic Microwave Background by Extragalactic Jets as a Probe of Their Matter Content". The Astrophysical Journal. 625 (2): 656–666. doi:10.1086/429558
Grupen, Claus; Cowan, Glen (2005). “Astroparticle Physics”. Springer. pp. 11–12. ISBN 978-3-540-25312-9.
Gurzadyan, V. G.; Ozernoy, L. M. (1979). "Accretion on massive black holes in galactic nuclei". Nature. 280 (5719): 214–215. doi. org10.1038/280214a0
Hainline, K. N., Hickox, R. C., Carroll, C. M., Myers, A. D., DiPompeo, M. A., & Trouille, L. (2014). “A spectroscopic survey of WISE-selected obscured quasars with the Southern African Large Telescope”. The Astrophysical Journal, 795(2), 124.
Hickox, R. C., & Alexander, D. M. (2018). “Obscured active galactic nuclei”. Annual Review of Astronomy and Astrophysics, 56, 625-671.
Kellermann, Kenneth (1992). "Variability of Blazars". Science. 258 (5079): 145–146. doi:10.1126/science.258.5079.145-a
Komberg, B. V. (1992). "Quasars and Active Galactic Nuclei". In Kardashev, N. S. (ed.). Astrophysics on the Threshold of the 21st Century. Taylor & Francis. p. 253
Kundt, W. (2014). “A Uniform Description of All the Astrophysical Jets”. Proceedings of Frontier Research in Astrophysics (FRAWS 2014), 25, 1-9.
Kraft RP; Vázquez S; Forman WR; Jones C; et al. (2003). "X-ray emission from the hot ISM and SW radio lobe of the nearby radio galaxy Centaurus A". Astrophysical Journal. 592 (1): 129–146. doi:10.1086/375533
Lambrides, E. L., Chiaberge, M., Heckman, T., Gilli, R., Vito, F., & Norman, C. (2020). “A Large Population of Obscured AGN in Disguise as Low-luminosity AGN in Chandra Deep Field South”. The Astrophysical Journal, 897(2), 160.
Masini, A., Hickox, R. C., Carroll, C. M., Aird, J., Alexander, D. M., Assef, R. J., ... & Murray, S. S. (2020). “The Chandra Deep Wide-field Survey: a new Chandra legacy survey in the Boötes field. I. X-ray point source catalog, number counts, and multiwavelength counterparts.” The Astrophysical Journal Supplement Series, 251(1), 2.
Matt, G., Fabian, A. C., Guainazzi, M., Iwasawa, K., Bassani, L., & Malaguti, G. (2000). “The X-ray spectra of Compton-thick Seyfert 2 galaxies as seen by BeppoSAX”. Monthly Notices of the Royal Astronomical Society, 318(1), 173-179.
Padovani, P., Alexander, D. M., Assef, R. J., De Marco, B., Giommi, P., Hickox, R. C., ... & Salvato, M. (2017). “Active galactic nuclei: what’s in a name?” The Astronomy and Astrophysics Review, 25(1), 1-91.
Parker, J., and Hickox, Ryan C. (2021) “X-ray observations of candidate active black holes in dwarf galaxies with the Chandra X-ray Observatory”. The Astrophysical Journal
Shapiro, S. L., & Teukolsky, S. A. (1983). “BookReview-Black-Holes White Dwarfs and Neutron Stars”. Journal of the British Astronomical Association, 93, 276.
Shields, G. A. (1999). "A Brief History of Active Galactic Nuclei". Publications of the Astronomical Society of the Pacific. 111 (760): 661. doi:10.1086/316378
Tiziana Di Matteo; et al. (2005). "Energy input from quasars regulates the growth and activity of black holes and their host galaxies". Nature. 433 (7026): 604–607. doi:10.1038/nature03335.
Wu, Xue-Bing; et al. (2015). "An ultraluminous quasar with a twelve-billion-solar-mass black hole at redshift 6.30". Nature. 518 (7540): 512–515. doi:10.1038/nature14241.