25 minute read
Ultrasonic deactivation of E. coli bacteria with a Zinc Oxide “hedgehog” sonocatalyst
Benjamin James
Barker College
Purpose: This paper investigates increasing the effectiveness of ultrasound as a method in bacterial inactivation using a ZnO ‘sonocatalyst’ (a catalyst activated through sound), as prior research has shown a high generation of free radicals on the ZnO surface under ultrasound. The ‘hedgehog’ crystalline morphology of ZnO in nanoparticle form was used based on observations of crystal implantation into bacterial cells through heavy mechanical stirring, pointing at the possibility that this catalyst may have multiple methods whereby it may deactivate bacteria. Approach: ZnO crystals with the “hedgehog” morphology were synthesized using a simple solution route suitable for the school laboratory environment. Diluted E.Coli K-12 broths with suspended ZnO crystals underwent ultrasonic irradiation, and were compared to control groups that were treated with ultrasound without the addition of crystals, and others that had crystals added without any ultrasonic irradiation, after all test groups were plated and incubated for 24h. Findings: While test groups that experienced no irradiation showed a minimal downtrend as catalyst concentration increased, test groups that received ultrasonic treatment with catalyst added displayed both a stimulating effect on the bacteria in smaller catalyst concentrations, and a significant increase in cell death for the highest concentrations. Proportionality between catalyst addition and cell deactivation could only be observed in the test group with the highest concentration of ZnO. Implications: These results indicate that future investigation into ultrasonic treatment of localized bacterial infections in vivo may be warranted to test the efficacy of this approach. Furthermore, investigation into the potential for cell growth stimulation using these treatment methods in tandem (for lower concentrations of the catalyst) can also be considered. Human applications may be limited to topical skin treatments due to the short range of penetration exhibited by high-frequency ultrasound. In vivo experimentation with ZnO “hedgehog” sonocatalyst may prove challenging due to nanoparticle spines unintentionally implanting into living subjects during application. Value: Ultrasonics are currently implemented in many medical and industry applications as an instrument sterilization method. Non-volatile catalysts such as ZnO may be viable in improving cleanliness overall, but also reducing sterilization time as under certain conditions, increased rate of cell death is observed when the catalyst is applied. Keywords: Ultrasonics, Sonochemistry, Oxide crystalline morphology Paper Type: Research Paper
Literature Review
Investigating novel forms of bacterial inactivation are on the rise due to worryingly high number of new bacterial strains with antibiotic resistance. Both photodynamic and sonodynamic (APDT and ASDT) therapies are currently under investigation, however ASDT has shown high efficacy in deep seated infections that block light-dependent treatments, especially with the addition of sonocatalysts (Costley et al, 2017).
Ultrasonic generation of cavitation bubbles has been known to induce chemical decomposition (Pétrier et al, 1997), and research of such processes upon destruction of organic material has been successful, but proposed mechanisms for damage are still debated (Zupanc et al, 2019, Gao et al, 2014). Higher observed deactivation rate on Gram-negative E.coli over Gram-positive S. mutans by Koda et al along with other cell-structure related research (Figure 1, summarized from Zupanc et al) supports a theory of increased resistance amongst Gram-positive bacterial strains to ultrasonic treatment. Figure 1 demonstrates this, displaying percentage of cells deactivated/logreduction (superscript ‘a’) values for Gram-negative (right) and -positive (left) bacteria when exposed to Low-Frequency Ultrasound (LFUS) in research conducted up to 2019. While Gram-negative results average to an ‘effectiveness’ average of 85.4%, effectiveness on -positive bacteria is only 73.5%. Notably, the genus Staphylcoccus from the Grampositive data is particularly resistant to LFUS, with a maximum effectiveness of only 40% (in reference to S. aureus, a species of growing concern due to the proliferation of MRSA, the highly dangerous
methicillin-resistant strain (Pasberg-Gauhl et al, 2014)). Factors regarding ultrasonic frequency, power, and sonication time have also been explored (Joyce et al, 2003), however only a relationship between sonication time and deactivation rate could be quantified with moderate certainty with ideal for LFUS at 25 mins (Koda et al, 2009).
GRAM-POSITIVE
Species Effectiveness (%) LFUS
Bacillus globigii 90
Streptococcus pseudintermedius 0.2a
Enterocuccus faecalis 75 Enterococcus avium 90
Lactobacillus acidophilus 0.6a Listeria innocua 3.2a
Mycobacterium species Strain (6PY1) 93
Staphylcoccus aureus
40 Staphylcoccus epidermidis 20/0.2a
GRAM-NEGATIVE
Species Effectiveness (%) LFUS
Cobetia marina 90
Enterobacter aerogenes
4.4a Haemophilus influenzae 99 Klebsiella pneumonia 90 Legionella pneumophila 20 Pseudomonas aeruginosa 2.7a Salmonella enterica 1a
Vibrio cholerao 90
Surface water coliforms 70.8
Figure 1: from Zupanc et al, 2019, displaying Low Frequency Ultrasound effectiveness in deactivation of Gram-positive AND Gram-negative bacteria from existing literature. Investigations implementing the use of radical scavengers demonstrated definitive evidence that reactive oxygen species (ROS) were generated in water under ultrasonic irradiation (Koda et al, 2009). Further experimentation with bacteria present indicated that ROS may be the primary cause for bacterial inactivation through the highly reactive radicals momentarily bonding with and oxidizing outer cell membranes through the cell wall, penetrating cell walls/membranes. Most notably, the ROS H2O2, (Hydrogen Peroxide) and ·OH radical are hypothesised to attack cell interior and membranes respectively (Rahman et al, 2010) through nonselective oxidation and destruction of cell components. The peptidoglycan cell wall layer present on Gram-positive bacteria that protects cell membrane is hypothesised to indicate their increased resistance against ROS (and hence ultrasound, Zupanc et al, 2019).
Zinc Oxide nanoparticles have been observed to show antibacterial properties on both Gram-positive and -negative species under ultrasonic irradiation in a broth. Mechanisms are debated (Figure 2, from Sirelkhatim et al, 2015), but most literature supports generation of harmful ROS on the ZnO molecule surface (Figure 3, top right) (Premanathan et al, 2011), Zn ion reactions with the cell membrane (Figure 3, bottom right) (Kasemets et al, 2009), or ZnO nanoparticle implantation into the cytoplasm via puncturing the cell wall (Figure 3, bottom left) (Rutherford et al, 2021). Interfacial surface potential difference between ZnO and the cell wall causing static discharge is also a proposed mechanism (Arkha et al, 2015). It is proposed that all mechanisms are valid, and which is most effective is shapedependent, as ZnO crystals come in a variety of morphologies (Sirelkhatim et al, 2015). Talebian et al (2013) demonstrate that high surface area, “flower” shaped crystal structures to be most effective in ROS generation under ultrasonic irradiation (Figure 4), due to a phenomenon amongst semiconductors called “electron-hole pairing”, an occurrence that is proportional to surface area. However, the “hedgehog” ZnO molecule has shown greater efficacy in deactivation through cell wall penetration using the needle-like spines on the molecule (Figure 3, from Rutherford et al, 2021). The high surface area of the “hedgehog” due to its many spines indicates that similar ROS generation processes to that of “flower” molecules may also occur. Furthermore, conventional ZnO nanoparticles have been identified to have higher efficacy against Gram-positive bacteria than Gram-negative
(Premanathan et al, 2011), which contradicts current literature and the trends in ultrasonic deactivation, and points to more research being required into this area. Thus, I will investigate how, under ultrasonic irradiation, the ‘hedgehog’ ZnO crystal may be viable in combining its cell wall penetration with surfacebased ROS generation, becoming an effective ‘sonocatalyst’ (a catalyst aided by ultrasound). This may increase its effectiveness in deactivating Gramnegative bacteria, which is traditionally more resistant to ZnO nanoparticles, but less resistant to ultrasound.
Figure 2: from Rutherford et al – Zinc Oxide “Hedgehogs” implanted in S. epidermidis bacteria. Figure 4: from Talebian et al – Zinc Oxide of “flower” shaped morphology
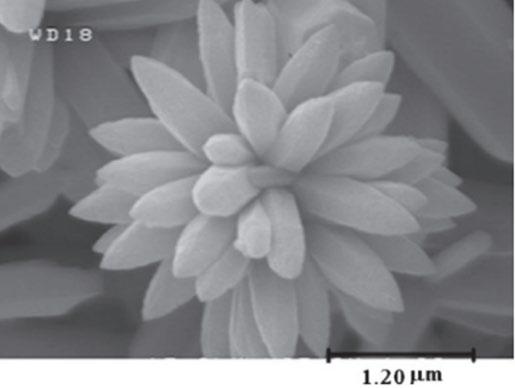
Scientific Research Question
Can the combination of ZnO “hedgehog” shaped nanoparticles and ultrasonic irradiation increase the rate of deactivation and growth inhibition of Gramnegative E. coli bacteria compared to either treatment alone?
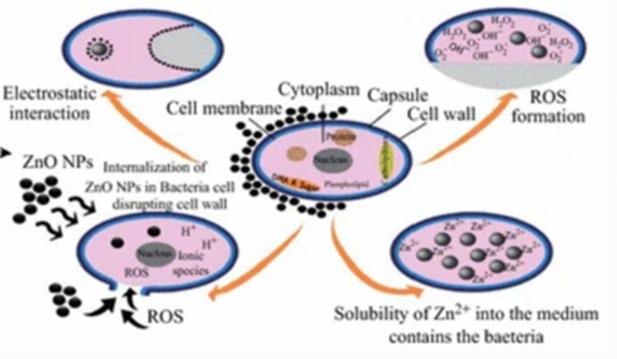
Figure 3 from Sirelkhatim et al – Mechanisms of bacterial deactivation through ultrasonic cavitation
Scientific Hypothesis
When compared to ultrasonic irradiation and nanoparticles unaccompanied, the addition of ZnO “hedgehog” nanoparticles while treating E. coli bacteria with ultrasound will increase the count of bacteria deactivated when ultrasonic irradiation is applied, and thus increase the inhibition of bacteria growth when compared to bacteria treated with nanoparticles but without ultrasound, and bacteria treated with irradiation alone.
Methodology
Zinc Oxide Preparation
As the Rutherford et al method of synthesising Zinc Oxide “Hedgehog” morphology crystals was not viable in a school laboratory environment, a “simple solution route” adopted from Zhang et al was used. In this case, their nomenclature for this morphology was deemed “prickly spherelike”. 25 mL of 0.5mol/L of a hydrated Zinc Acetate (ZnAc2) solution and 5mol/L NaOH were mixed dropwise with a 1:1 volume ratio to prepare the Zn(OH)4 -2 precursor. 1.2 mL of Zn(OH)4 -2 solution and 13.2 mL of ethanol were mixed under consistent stirring, then transferred into the autoclave which was pre-lined with Teflon baking sheets. Taking time considerations from Zhang et al’s investigations on time the solution spent in the autoclave vs the resulting crystalline structure definition, 2 full 10-cycle runs were made in the autoclave for a total of 50 minutes at 120°c. The precipitate suspended within the remaining ethanol in the beaker was then collected and washed 4 times with absolute ethanol and once with dH2O through filter paper. The precipitate was then collected through centrifugation to separate solid components. The supernatant was placed in sealed side-arm flask underneath a heating element, and plastic tubing was run from the hose barb to the air inlet of a hand-pump vacuum ejector. Rutherford et al observed extreme hygroscopic effects which caused ‘clumping’ in ZnO crystals, leading to difficulties in imaging as clumped oxide crystals amplify conductive effects. Dehydrating the precipitate under vacuum pressure minimised this effect, and the collected crystals were transferred into a glass desiccator with silica gel desiccant for long-term storage.
Ultrasonic Testing
The ultrasonic testing phase involves treating E. coli broth with three increasing concentrations of ZnO “hedgehogs” (including concentration of zero) both with ultrasonic irradiation and with no treatment, and comparing kill rates of each test group.
A 250mL stock solution of E. coli was divided into 50mL conical flasks for one ultrasonic treatment without ZnO, and one batch per ZnO concentration (100μg/mL and 1000μg/mL), along with 3 corresponding 50mL flasks to receive ‘static’ treatment (control), which involved resting away from the ultrasonic bath (‘static’ in this case refers to the flasks experiencing no irradiation, and does not refer to any interactions involving electrostatic forces). Glassware was sterilised in an autoclave for 25 minutes with 10 total cycles at 121°C. Bacteria was transferred to conical flasks under a convection flame in a fume hood, and flasks that required ultrasonic treatment were secured to the outer walls of the ultrasonic bath with electrical tape as they were naturally buoyant and would otherwise float. When required, Zinc Oxide was poured into the flasks after each was placed on the test bench or in the ultrasonic bath, to minimise dynamic interactions with bacterial cultures that could occur during transport to the bath/test bench, and cause bacterial deactivation (recommendation of Rutherford et al’s method). Flasks were covered in aluminium foil to minimise interactions of ultraviolet light with bacteria which can also catalyse deactivation (as per Costley et al), and treatment (either sonication or at rest on the test bench in the case of controls) occurred for 25 minutes.
Each test had a control alongside the irradiation (50mL flask with no ZnO and no ultrasonic treatment), with results from each batch scaled to their respective controls to account for differing concentration between the E. coli stock solutions. Adopted from Rutherford et al, the plating method was adjusted to decrease colony density to improve the definition of the lawn for counting. A 1:100 dilution series using .1mL of treated culture and 9mL .9% NaCl was performed for each experimental batch post-treatment. 1 drop of diluted culture was added to agar plates, with 3 duplicates per test group. Plates were incubated at 36.5°C and the number of viable colonies was recorded 24 hours after the time of sonication using a digital colony counter.
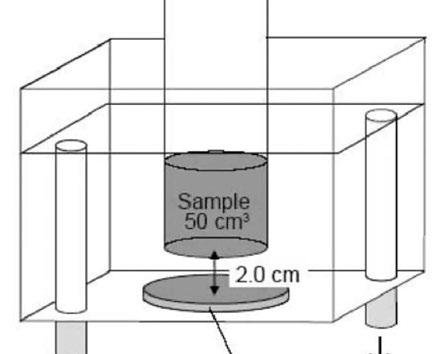
Figure 5: Example sonication setup in ultrasonic bath, with the beaker submerged in water, kept from bath floor by aluminium cage (not present in diagram)
Material Characterisation (Scanning Electron Microscopy)
ZnO crystals required characterisation to ensure that the correct morphology had been synthesised. Method for SEM imaging was further adopted from Rutherford et al, with their images takes as a reference for imaging scale and as a general guide on the intended image. ZnO was poured onto carbon tape which was stuck to an SEM stub. Particles were sputter coated with 2nm Gold layer in CCU-010 HV Compact coating unit to reduce charging. SEM images were taken in Zeiss Sigma HD VP FEG SEM on high vacuum setting, with 5kV accelerating voltage with Everhart-Thornley (SE2) and In-lens Secondary electron detectors.
Statistical Analysis
Viable bacteria concentration (with units of “colony forming units” per millilitre, cfu/mL) was calculated through multiplication of colony number (averaged between the four total 1cm2 counted areas for each of the three replicates) by the dilution factor (1:100), and dividing the result by the analysed area of the agar plate (1cm2). A one-tailed student’s t-test (α=0.05) was used to analyse whether the viable bacteria concentrations of the treatments with Zinc Oxide Hedgehogs were significantly different from the control distribution. Conducting f-tests each testing group (in order to determine whether certain plates were significantly different from the expected variance) was deemed redundant due to low number of replicates (3) that resulted in unreliable variance calculations
Viable cell counts post-treatment
Viable cell concentration calculations were made using the average bacterial count value over 1cm3 for each of the three replicates for all six test groups. Error bar maximum and minimum bounds were found by calculating residual error for each test group.
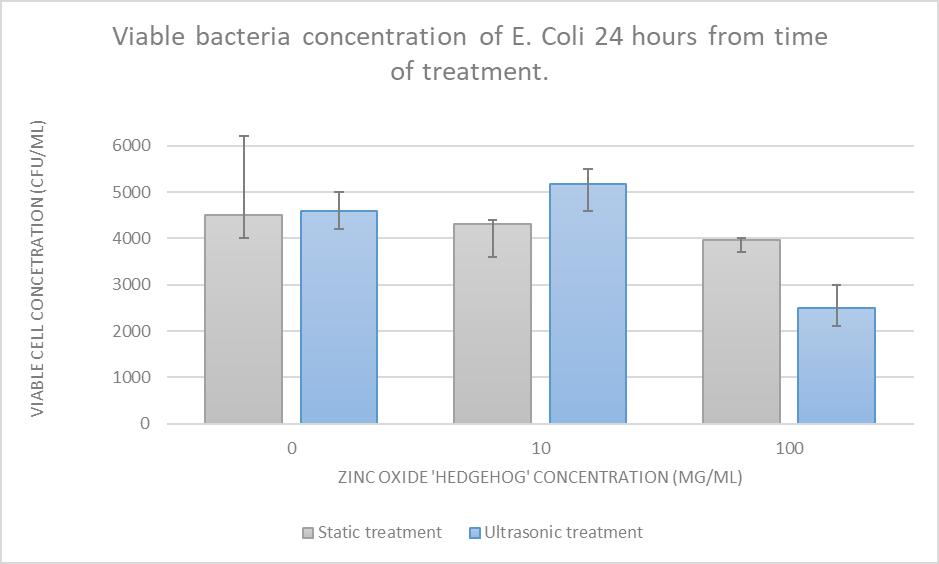
Figure 6: Viable cell concentration vs ZnO concentration, comparing ultrasonic and static treatment. Data from Table 1.
Table 1: Bacterial counts for each 1x1cm sample plate.
Bacterial counts
Test Group 1 2 3 Average
Control 43 50 52 45
Ultrasonic treatment + ZnO 46 55 54 51.7
Control + ZnO 42 36 41 39.7
Ultrasonic treatment + ZnO 24 30 21 25
The “Static treatment” coefficient of determination (R2 value) of 0.9796 demonstrates an acceptable correlation for increased catalyst concentration to be directly proportional to cell death, however the error margins for the 0 mg concentration test group lead to significant uncertainty to be attached to this conclusion (to be discussed). Regardless, a negative trend in some form is qualitatively observable from Figure 6.
“Ultrasonic treatment” received a coefficient of determination of 0.5586, which is low enough to conclude that no trend is observed in this data set.
Imaging ZnO morphology
Structural similarities to the spines apparent in Figure 7 and classroom-synthesised samples from SEM images indicate the developed morphology being “prickly spherelike”. Comparisons in Figure 8 (and the lower image of Figure 7) provide qualitative analysis of the physical similarities between “hedgehogs” using Zhang et al’s simple solution route and those developed in original literature. Furthermore, parallels within Figure 7 demonstrate that 100nm long spines are present in both samples, while the spine length in Figure 8 differs by a factor of 10. This outlines that while similar in visual structure and effect (see Figure 6), the competing synthesis methods do result in a difference in scale.
In Figure 8, a ”clumping” effect can be observed amongst the crystals developed in this project. While visually Rutherford et al developed ZnO samples that were limited to a low amount of rod-like spines that joined in larger clumps (see Figure 3), defined spines cannot visually be distinguished in Figure 8 (left) due to the frequency of spines. However, clumps in the 500-1000nm range are observed in both Rutherford et al, Zhang et al, and this research, further confirming the similarities in crystal structure between the simple solution route and the Rutherford et al methodology.
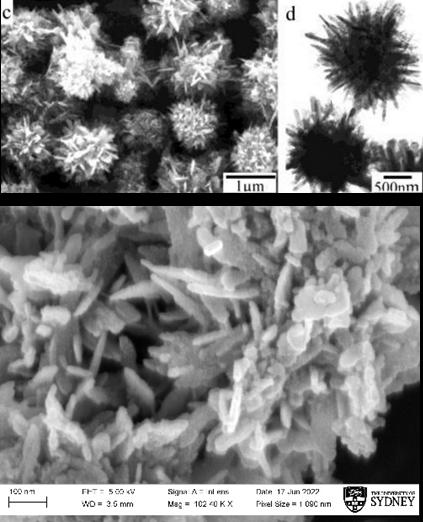
Figure 7: Comparison between Zhang et al’s “prickly spherelike” morphology and nanoparticles developed in classroom environment.
Figure 8: Comparison to Rutherford et al’s “hedgehog” morphology.
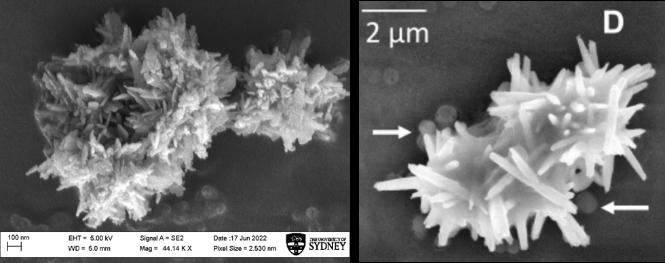
Statistical Results
One-tailed t-tests for each concentration of ZnO were carried out. The 10 mg and 100 mg test groups that experienced ultrasound were compared to both the corresponding “static interaction” tests, as well as the ultrasonic treatment test for 0 mg. This allowed both aspects of the hypothesis, being a reduction in bacterial growth in comparison to no treatment and ultrasonic irradiation alone, to be analysed.
The results for the 0 mg group were found to not be significantly different, and thus no correlation could be observed between ultrasonic irradiation alone and increased bacterial deactivation. For the 10 mg test group, while the statistical significance in comparison to the expected mean was acceptable (t = 2.600, p = 0.0450 compared to ultrasound alone, t = 3.5301, p = 0.0242 compared to static interaction). Compared to ultrasonic treatment alone and static
interaction (no ultrasonic treatment), the 100 mg test group (t = 4.5383, p = 0.0105) was deemed statistically significant (a = 0.05).
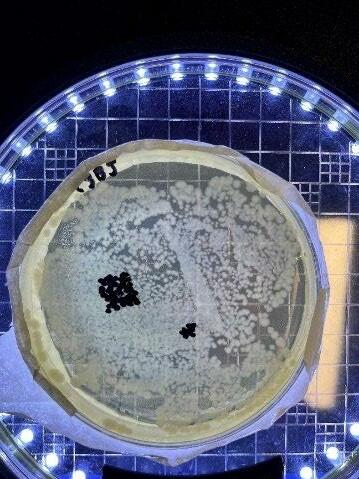
Figure 9: One plate for the 0 mg, “static interactions” group. Left side of plate demonstrates the density issue faced when counting plates for this group
No correlation could be found between increased bacterial deactivation and cell death for the 0 mg test group. This somewhat contradicts the findings of Koda et al and others who found success in bacteria death without sonocatalyst implementation. Systematic error in terms of application of ultrasound can be disregarded, as observations of irradiation with ZnO crystals suspended in the broth found that after treatment, larger crystals were no longer visible. It can be inferred that ultrasound was imparting mechanical waves into the water as such dispersing of larger particles is a direct result of mechanical vibrations breaking apart higher-mass structures (Joyce et al, 2003). A conclusion can be drawn due to the qualitatively immeasurable density on the agar plate that the significance calculated is understating the true difference of these results (Figure 9), hence the extended upper bound of the error bar for the 0 mg “static interactions” test group. Regardless, both data points act simply as controls by which to compare the test groups with the catalyst added.
For the 100 mg test group the p-value for both t-tests was lower than the alpha value, thus the hypothesis that ZnO “hedgehog” crystals will increase the inhibition of bacterial growth compared to irradiation alone and no treatment is supported.
The 10 mg test group, however, (conversely to the hypothesis) was the highest of all groups in bacterial growth. Analysis by Rutherford et al after observing a similar effect at this concentration concluded that Zinc ions in proximity to cell wall can influence cell development. Hara et al outline that Zn transportermediated ions of Zinc, labelled “Zinc signals”, manage signalling pathways that control growth factors within the cell. However, the properties of the cell designed to detect these signals may be sensitive enough to detect them beyond the bounds of the cell interior. The effects of free Zinc ions that separate from the ZnO crystal and send “phantom signals” to the cell by existing within a certain proximity of the cell membrane may initiate growth at a rate that outweighs the bacterial deactivation through ROS generation, promoted by ultrasonic irradiation.
While mechanisms of bacterial deactivation are beyond the scope of this research, the theory of Arakha et al can be further considered due to observed results. SEM image resolution was detrimentally affected by the ZnO “clumping” effect, as larger particles demonstrate a conductive effect when hit with the electron beam. It can be inferred from this increased conductivity that static interactions between the outer, negatively charged layers of the cell and the ZnO clumps may, as proposed by Arakha et al, have contributed to cell death in this instance.
“Clumping” of ZnO can be attributed to an experimental issue regarding pressurising the sidearm flask when dehydrating the supernatant after centrifugation. When releasing the pressure on the vessel to open it and collect the crystals, water that had precipitated but condensed in the plastic tubing was pulled back into the flask, causing much of the ZnO to reabsorb moisture (confirmed when the powder turned a milk white when a scraper was ran along it). This required the flask to be reheated to remove this excess water, but as the absorbed water causes the crystals to bind together under surface tension, this reheating process may not have completely dehydrated the samples. Regardless, observations made earlier on larger ZnO particles breaking down under the mechanical force of the ultrasonic waves demonstrates that larger “clumped” particles are not detrimental to results, as they were broken down by sonication irrespective of size.
This research is limited by the limited yield of ZnO that could be collected in a classroom laboratory environment. While Zhang et al’s method by which this synthesis was derived called for a low volume Teflon-lined autoclave, we opted to line the beaked containing the solution with Teflon instead, as this
beaker was not an available resource. The ZnO “prickly spherelike” crystals precipitate from solution within the atmosphere inside the autoclave, rather than simply be confined to the original beaker. A lower yield of precipitate was collected after the process was complete, and much of this lost ZnO can be attributed to the Teflon lining of the beaker both not forming an airtight seal, and collection of ZnO powder from the Teflon proving difficult due to the adhesive nature of the precipitate to such surfaces. In the research of Zhang et al, the entire yield could have been salvaged as the precipitate ZnO was contained to the walls of the autoclave. This lower yield warranted less replicates and thus lower reliability, as well as only three (including controls) concentrations of the catalyst being tested, while four concentrations may have provided a better chance at a final trend appearing amongst the results.
The results of this research have implications in many directions and fields. Comparisons between the traditional method of “hedgehog” synthesis and the simple solution route that results in “prickly spherelike” morphology can be made in terms of bacterial deactivation rate under stirring and ultrasound, as the difference in spine size that was observed in this paper may affect both mechanisms of cell death and effectiveness.
In terms of applications directly to humans, the lower penetration of high-energy ultrasound into the body may prevent this treatment method for internal conditions, however deep-seated bacterial skin infections may benefit from this combination of treatment methods as an alternative to antibiotics. However, the nanoparticle size (and particularly the design of this morphology, which is intended to implant itself into cells) may cause further harm due to implantation within and around the body of a patient.
Furthermore, sonocatalysts are still in their research infancy due to lack of popularity and necessity within current applications of sonication in industry settings besides sterilisation. However, attempting to demonstrate semiconductors as a viable addition to ultrasonic cleaning processes may change this sentiment due to the high scale fabrication ability of many semiconductors due to existing production infrastructure for technological applications, in comparison to more chemically complex catalysts. Moreover, this combination of treatment methods may hypothetically reduce sterilisation time as bacteria killed over a constant time was found to increase, thus inferring that bacterial kill rate also increased. Adding to the existing knowledge bank of ultrasonic treatment methods by testing the efficacy of novel methods by which to increase effectiveness may allow for future progress to be made beyond sterilisation and towards in vivo applications. As antibiotics continue to face higher antimicrobial resistance, ultrasonics may be a necessary alternative that we must develop from such knowledge.
Finally, the most intriguing result from this paper is the stimulating effect that lowered concentrations of the catalyst had on the bacteria when ultrasound was applied. Although growth stimulation of harmful bacteria cells is not warranted in most cases, potential applications of “phantom signalling” increasing the growth rate of bacterial cultures should be investigated. Searching for and minimising this effect in certain environments may reduce the chance of bacterial infection by decreasing its growth rate.
Conclusion
This work investigated the efficacy of increasing the bacterial deactivation effectiveness of ZnO nanoparticles of “hedgehog” morphology by using the nanoparticles as a sonocatalyst when bacteria is irradiated with ultrasound. Using a school laboratory appropriate synthesis method entitled the ‘simple solution route’, nanoparticles were formed and added to diluted E. coli broths in increasing concentrations. Control groups that experienced no ultrasonic treatment were compared to groups that received 25 minutes of sonication. Morphology was confirmed to structurally replicate that of the “hedgehog” crystal structure by analysis through SEM imaging at 5001000nm range. For larger concentrations of ZnO, proportionality between catalyst addition and increased bacterial deactivation in comparison to the control group was supported. For the 10 mg concentration, a stimulating effect was observed in that the catalyst promoted cell growth in comparison to the control, which aligned with similar results found in previous experimentation. Thus, the efficacy of ZnO as a sonocatalyst modulates by particle concentration, while this particular morphology was found to significantly increase cell kill rates at higher volumes relative to the bacterial solution. There is a potential, therefore, for this combination of treatment techniques to promote applications of ultrasonic treatment against bacteria in more environments where sensitive equipment or living subjects are involved and complete sterilization is imperative. This may include medical and industry applications, as well as in vivo treatments of topical bacterial
infections, however risks associated with nanoparticle use are present. Similarly, the accelerated bacterial growth observed when the catalyst was added at low concentrations may promote investigation of “phantom signaling” as a cause for bacterial dominance in some environments.
Acknowledgements
I would like to thank Dr Terena Holdaway-Clarke for her support through the duration of this project, especially her guidance regarding microbial research and technique, as well as the equipment and time she has dedicated to the progress of this paper. I would also like to thank the Barker College laboratory staff for their assistance in ordering and obtaining all of the necessary materials for my project, as well as providing laboratory spaces.
I would also like to acknowledge the work of David Rutherford, and his helpful correspondence throughout this project that assisted me in the production and storage techniques used for aspects of this project that were adopted, but not directly addressed, in his prior research.
Finally, I would like to extend gratitude to Ashalatha and the team at Sydney Microscopy and Microanalysis at the University of Sydney for providing the opportunity to utilise their SEM equipment, as well as their assistance in preparing samples and conducting the imaging. The value of these images to my project is immeasurable and their cooperation in organising and allowing me to make use of their facilities is greatly appreciated.
References
Pétrier, C., & Francony, A. (1997). Ultrasonic waste-water treatment: incidence of ultrasonic frequency on the rate of phenol and carbon tetrachloride degradation. Ultrasonics sonochemistry, 4(4), 295-300.
Costley, D., Nesbitt, H., Ternan, N., Dooley, J., Huang, Y. Y., Hamblin, M. R., ... & Callan, J. F. (2017). Sonodynamic inactivation of Gram-positive and Gram-negative bacteria using a Rose Bengal–antimicrobial peptide conjugate. International journal of antimicrobial agents, 49(1), 31-36. Zupanc, M., Pandur, Ž., Perdih, T. S., Stopar, D., Petkovšek, M., & Dular, M. (2019). Effects of cavitation on different microorganisms: The current understanding of the mechanisms taking place behind the phenomenon. A review and proposals for further research. Ultrasonics sonochemistry, 57, 147-165. Gao, S., Lewis, G. D., Ashokkumar, M., & Hemar, Y. (2014). Inactivation of microorganisms by low-frequency high-power ultrasound: 1. Effect of growth phase and capsule properties of the bacteria. Ultrasonics Sonochemistry, 21(1), 446-453.
Piyasena, P., Mohareb, E., & McKellar, R. C. (2003). Inactivation of microbes using ultrasound: a review. International journal of food microbiology, 87(3), 207-216.
Koda, S., Miyamoto, M., Toma, M., Matsuoka, T., & Maebayashi, M. (2009). Inactivation of Escherichia coli and Streptococcus mutans by ultrasound at 500 kHz. Ultrasonics sonochemistry, 16(5), 655-659.
Rahman, M. M., Ninomiya, K., Ogino, C., & Shimizu, N. (2010). Ultrasound-induced membrane lipid peroxidation and cell damage of Escherichia coli in the presence of nonwoven TiO2 fabrics. Ultrasonics sonochemistry, 17(4), 738-743.
Rutherford, D., Jíra, J., Kolářová, K., Matolínová, I., Mičová, J., Remeš, Z., & Rezek, B. (2021). Growth inhibition of gram-positive and gram-negative bacteria by zinc oxide hedgehog particles. International Journal of Nanomedicine, 16, 3541.
Zhang, J., Sun, L., Yin, J., Su, H., Liao, C., & Yan, C. (2002). Control of ZnO morphology via a simple solution route. Chemistry of Materials, 14(10), 4172-4177.
Joyce, E., Phull, S. S., Lorimer, J. P., & Mason, T. J. (2003). The development and evaluation of ultrasound for the treatment of bacterial suspensions. A study of frequency, power and sonication time on cultured Bacillus species. Ultrasonics sonochemistry, 10(6), 315-318.
Sirelkhatim, A., Mahmud, S., Seeni, A., Kaus, N. H. M., Ann, L. C., Bakhori, S. K. M., ... & Mohamad, D. (2015). Review on zinc oxide nanoparticles: antibacterial activity and toxicity mechanism. Nano-micro letters, 7(3), 219242.
Premanathan, M., Karthikeyan, K., Jeyasubramanian, K., & Manivannan, G. (2011). Selective toxicity of ZnO nanoparticles toward Gram-positive bacteria and cancer cells by apoptosis through lipid peroxidation. Nanomedicine: Nanotechnology, Biology and Medicine, 7(2), 184-192.
Kasemets, K., Ivask, A., Dubourguier, H. C., & Kahru, A. (2009). Toxicity of nanoparticles of ZnO, CuO and TiO2 to yeast Saccharomyces cerevisiae. Toxicology in vitro, 23(6), 1116-1122.
Talebian, N., Amininezhad, S. M., & Doudi, M. (2013). Controllable synthesis of ZnO nanoparticles and their morphology-dependent antibacterial and optical properties. Journal of Photochemistry and Photobiology B: Biology, 120, 66-73.
Pasberg-Gauhl, C. (2014). A need for new generation antibiotics against MRSA resistant bacteria. Drug Discovery Today: Technologies, 11, 109-116.
Zhang, J., Sun, L., Yin, J., Su, H., Liao, C., & Yan, C. (2002). Control of ZnO morphology via a simple solution route. Chemistry of Materials, 14(10), 4172-4177.
Arakha, M., Saleem, M., Mallick, B. C., & Jha, S. (2015). The effects of interfacial potential on antimicrobial propensity of ZnO nanoparticle. Scientific reports, 5(1), 110.
Hara, T., Takeda, T. A., Takagishi, T., Fukue, K., Kambe, T., & Fukada, T. (2017). Physiological roles of zinc transporters: molecular and genetic importance in zinc homeostasis. The Journal of Physiological Sciences, 67(2), 283-301.